For several months now, I have been writing about process controls role in addressing the global energy crisis. In this article, I will lay out in some detail my approach to solving the problem through combining the technologies of the electrolyzer and the fuel cell to make solar energy available, both during the day and at night.
During the last century, the world population quadrupled. During the last 50 years, the global energy consumption has also quadrupled, and on top of that, during the last five years, the wholesale price of energy also quadrupled.
In addition to running out of fossil fuel deposits, the carbon dioxide concentration of the atmosphere has also reached the highest level in 650,000 years and is rising faster than ever. This will cause natural disasters, while half a billion people are already starving.
It is time to realize that the market forces cannot solve the energy crisis, because rising prices cannot increase the sum total of the size of the global deposits, they can only motivate the exploitation of more and more damaging sources. The energy crisis has no military solution either! More nuclear missiles will not reduce carbon emissions nor will they increase the remaining fossil or nuclear deposits. They will only waste our economic resources and consume the funds that are needed to solve the crisis.
Yet, solar energy is free, clean and plentiful. Therefore, we must accept that the solution is new technology. We should also realize that advanced process control will play a key role in this transition.
In the May issue (Green Energy Can Stop Recession] I mentioned that I am publishing a book (See Figure Here), describing this solution by providing and explaining the detailed design and controls of the worlds first, full size (1,000 mW) solar-hydrogen power plant. In the May issue I also referred to my invention of the reversible fuel cell (RFC), the Lipták cell. The reason why the RFC is so important is because it makes solar energy available night and day, continuously.
In the May issue I concentrated on the electrolyzer and just briefly mentioned that its operation can be reversed to operate as a fuel cell. I also described how process control can reduce the size, weight and, therefore, the cost of these reversible fuel cells (RFC) from todays price of about $3,000/kW to about $250/kW by the steps I will briefly describe below and in more detail in my book.
Fuel Cells
The traditional fuel cell generates electricity, heat and distilled water by reacting hydrogen with air (oxygen). In the space shuttle and in the space station, both electricity and water are generated as the stored hydrogen is oxidized in it. Fuel cells have been used not only in space exploration, but also in submarines (because they generate no noise or vibration). They have also been used to recover the energy from methane, which can be generated by wastewater and garbage dumps or from natural gas and more recently been also used in buses, automobiles and other vehicles as alternatives to the internal combustion engine.
The fuel cell consists of an electrolyte, which is sandwiched between two electrodes (See Figure). They come in many designs, including alkaline, DMFC (direct methanol fuel cell), MCFC (molten carbonate fuel cell), PAFC (phosphoric acid fuel cell), PCFC (protonic ceramic fuel cell), PEM (proton exchange membrane), RFC (reversible fuel cell), SOFC (solid oxide fuel xell) and ZAFC (zinc-air fuel cell), etc. The electrolytes can be acidic or alkaline and liquid, solid or solid-liquid composites.
PAFCs are the first generation of the mature designs. They are often used in larger vehicles and buses. These are medium temperature 200 °C (about 400 °F) units, generally available in the 60kW to 200 kW size range. They can be up to 85% efficient when used to generate both electricity and heat, but are only about 50% to 60% efficient when generating electricity only. They are large, heavy and cost about $4,000/kW.
The first fuel cell design used in the U.S. space program was that of the low-temperature alkaline fuel cell (AFC). Its disadvantages include that it is subject to carbon monoxide poisoning, is expensive and its operating life is short. The AFC electrodes are made of porous carbon plates laced with catalyst. The electrolyte is potassium hydroxide. At the cathode, oxygen forms hydroxide ions that are recycled back to the anode. At the anode, the hydrogen gas combines with hydroxide ions to produce water vapor and electrons that are forced out of the anode and produce the electric current.
Single cells are rarely able to produce enough power as is required by commercial applications. Therefore the cells are combined into stacks. Commercial stacks frequently have more than a hundred and sometimes as many as 400 cells. Todays fuel cells are expensive. In the 2 mW to 4 mW size range they cost from $3000/kW to $4,000/kW. According to the U.S. Department of Energy, this cost range compares to a diesel generator cost range of $800 to $1,500 per kilowatt, and a natural gas turbine, which can be purchase for $400 per kilowatt or even less. Therefore, reducing the fuel cell cost to $250/kW would make it competitive for virtually every type of power application.
This year, in Folsom, Calif., Altergy Systems started up the worlds first automated assembly line for the manufacturing fuel cells. The Department of Energy and the National Renewable Energy Laboratory estimated that if the production volume of proton electrolyte membrane (PEM) fuel cells reached 500,000 units per year, their unit cost could drop by an order of magnitude.
The efficiency of single-cycle fuel cells range from 47% to 50%; the efficiency of combined-cycle fuel cells is about 60%, and if the generated heat is also recovered (in the form of hot water or steam), their total efficiency could be around 80%. (The efficiency of gasoline engines is around 25%, of nuclear power plants about 35% and of sub-critical fossil fuel power around plants 37%.)
In the PEM design, the membrane electrode assembly consists of the anode and the cathode that are provided with very thin layers of catalyst bonded to either side of a proton exchange membrane. With the help of this platinum catalyst, the hydrogen at the anode splits into a proton and an electron while oxygen enters at the cathode. When hydrogen reaches the catalyst layer, it separates into protons (hydrogen ions) and electrons. The protons (See Figure) pass through the electrolyte while the free electrons are conducted in the form of a usable electric current- through an external circuit.
At the cathode, the electrons combine with the oxygen in the air and with the hydrogen protons that migrate through the proton exchange membrane to produce water and heat. Air flows through the channels to the cathode. The electrolyte can be a solid polymer, while the electrodes can be made of porous carbon and the catalyst of platinum. To obtain the desired amount of electrical power, individual fuel cells are combined to form fuel cell stacks. Increasing the number of cells in a stack increases the voltage, while increasing the surface area of the cells increases the current. It takes only a few seconds for cold fuel cells to start producing electricity.
The Reversible Fuel Cell (RFC)
In my solar-hydrogen demonstration power plant design, the functions of the electrolyzers and of the fuel cells are combined into single units, which can operate in either mode. These reversible fuel cells (RFCs) during the day will operate in the electrolyzer mode (See Figure Here), converting solar energy into chemical energy (hydrogen), while at night they will switch into their fuel cell mode and will convert the chemical energy stored in hydrogen back into electricity.
By keeping the pressures identical on the two sides of the membrane, these dual-purpose cells can be made light and thin. They also will use only half as much expensive platinum catalyst, and therefore, be much less expensive.
It takes the same amount of energy to split water into hydrogen and oxygen as the energy obtained when hydrogen is oxidized into water. The only difference is that electrolysis increases the entropy, and, therefore, not all the energy needs to be supplied in the form of solar electricity because the environment contributes 48.7 kJ/mole of thermal energy. Inversely, when the RFC is operated in fuel-cell mode, part of the energy in the hydrogen fuel is released as heat. Therefore, the electrolysis mode of operation (Shown in blue in Figure) requires heat, and the fuel cell mode (Shown in red in Figure) releases heat.
In a solar-hydrogen power plant, when excess solar energy is available, the RFC is switched into the electrolyzer mode to split water into hydrogen and oxygen. The hydrogen is collected and is either liquefied or compressed to high pressure (about 1,000 bars = 15,000 psig) and sent to storage.
Whenever solar electricity is insufficient, the RFC is switched into the fuel-cell mode in which the oxidation of one mole of hydrogen will generate 237.1 kJ/mole of electrical energy plus 48.7 kJ/mole of thermal energy. This waste heat also can be used for heating buildings or for preheating boiler feed water.
Controlling the RFC
The role of process control is critical in operating the RFCs. The complexity of the control challenge can be appreciated if we view a stack of 400 RFC cells as 400 pumps operating in parallel, and we realize that switching the RFC from one mode to the other is like switching a chemical reactor from one product to another. Fortunately, the switchover doesnt need to be fast, but once the RFC is in operation, its time constant is very shorta matter of seconds.
In addition to the electric controls that connect the RFC to the grid and convert the direct current to alternating current, a massive quantity of measurements and control algorithms are required. These include the switching between the heating and cooling modes as the RFC operation is reversed. These loops require high rangeability and fast, accurate temperature controls in both modes. The pressures of the oxygen and hydrogen streams entering (in the fuel-cell mode) or leaving the RFC (in the electrolyzer mode) also must be controlled carefully. The oxygen and hydrogen pressures also require accurate controls, because these pressures have to be identical, so that the proton exchange membrane (PEM) diaphragms of the fuel cells will not be exposed to excessive pressure differences.
In addition, the loads (the rates of hydrogen or electricity generation) need to be controlled. These loads are either determined by the availability of excess solar electricity (electrolyzer mode: blue, Figure 1) or by the electricity demand (Fuel-cell mode: red, see figure). Maintaining the load in both modes requires fast and accurate flow controls.
In the fuel-cell mode, the hydrogen fuel flow has to be controlled, while in the electrolyzer mode, the flow of the distilled water supply is one of the key manipulated variables. Controls are also needed to direct the generated distilled water to its destination (FC mode) and to send the generated oxygen to its destination (electrolyzer mode). The destination for distilled water can be the drinking water system, while the destination for the generated oxygen can be the air supply to a fired heater or boiler, if such a unit exists on the site (to increase efficiency by increasing the oxygen concentration of the air).
The instruments used will have to be mass-produced, miniaturized, accurate and inexpensive. The control algorithms have to be state-of-the-art (because my system is not yet patented, here I am not describing the algorithms).
In short, process control will play a key role in the transition from the present fossil/nuclear economy to the inexhaustible and free solar-hydrogen economy of the future. I am sure that our process control profession will meet these challenges, and thereby will not only play a key role in this third industrial revolution, but also will gain the respect it deserves as the most important field of engineering.
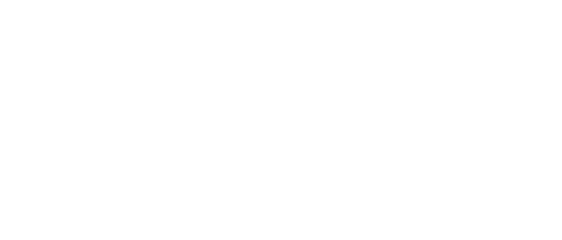
Leaders relevant to this article: